Full Text
Introduction
Nanotechnology was first proposed by the Nobel Prize winner Richard Feynman in 1959 [1]. The biomedical application of nanoparticle is a rapidly developing area of nanotechnology that raises new possibilities in the diagnosis and treatment of human cancers. On the metric scale, a nanometer is one-billionth of a meter. Nanoparticles are structures ranging in size from 1 to 100 nm (Figure 1). Nanoparticles show unique size-dependant physical and chemical properties, which can be optical, magnetic, catalytic, thermodynamic, and electrochemical [2]. These particles have great potential for clinical use, and the National Institute of Health (Bethesda, MD, USA) has referred to this area as nanomedicine.
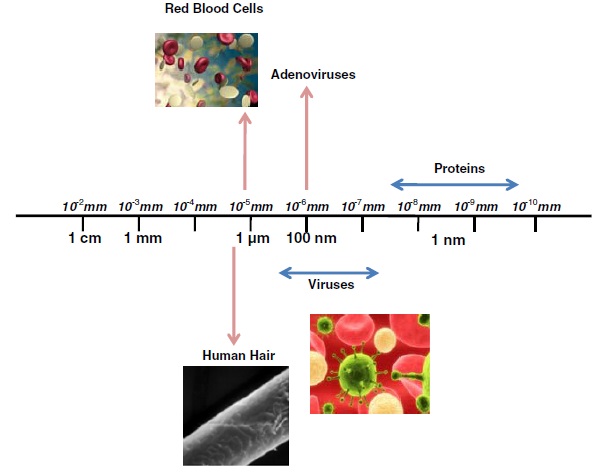
Figure 1: particle size comparison.
Most cancer therapeutics are small drug molecules that after being ingested or injected into the bloodstream can easily diffuse through vascular pores and the extracellular matrix to reach tumors. Complex therapeutics that involve drug delivery mechanisms or imaging moieties have tended to be much larger. While the exact size of molecules that can easily transverse vascular pores from the bloodstream and reach tumor tissue is unclear, it is probably limited to the size of proteins (<20 nm). Nanoparticles have many potential benefits for diagnosing and treating metastatic cancer, including the ability to transport complex molecular cargoes to the major sites of metastasis, such as the lungs, liver and lymph nodes, as well as targeting to specific cell populations within these organs [3]. The rapid developments in nanostructured materials and nanotechnology will have profound impact in many areas of biomedical applications including delivery of drugs and biomolecules, tissue engineering, detection of biomarkers, cancer diagnosis and cancer therapy.
Different nanotechnology-based nanocarrier systems Based on nanotechnology, nanocarriers synthesized from organic and inorganic materials have been developed, such as liposome, micelles, quantum dots, gold nanoparticles, magnetic nanoparticles, carbon nanotubes and dendrimers [4, 5] (Figure 2). They have shown great potential in cancer therapy by enhancing the performance of medicines and reducing systemic side effect in order to gain therapeutic efficiency.
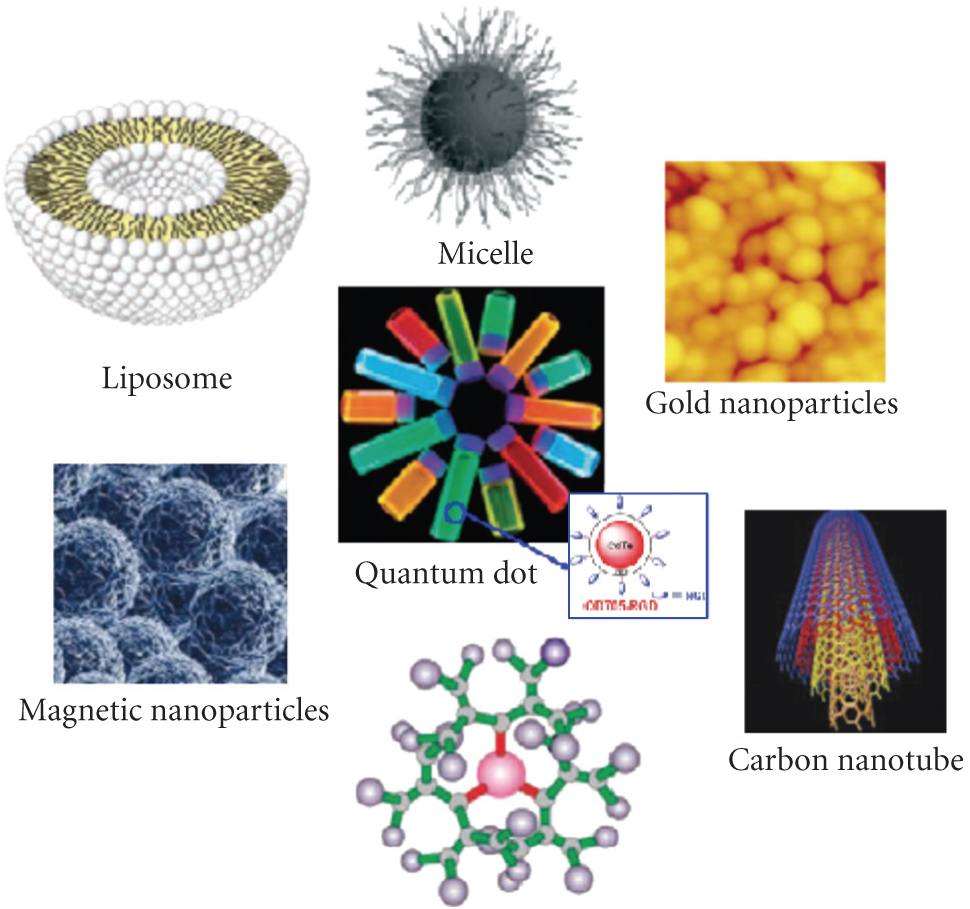
Figure 2: Examples of nanomaterials and nanocarrier systems (W. Cai et al. [4, 5]).
Liposome: A liposome is a tiny bubble (vesicle), made out of the same material as a cell membrane. Liposomes can be filled with drugs, and used to deliver drugs for cancer and other diseases. Thermosensitive magnetoliposomes (TMs) encapsulated with methotrexate (MTX) prepared by reverse-phase evaporation can achieve a good magnetic targeting effect and rapid drug release in response to hyperthermia, which implies their great potential in cancer therapy [6]. Healthy fibroblast cells and breast cancer (MCF-7) cells were treated with either free phthalocyanine or phthalocyanine bound to either gold nanoparticles or encapsulated in liposomes [7]. A human breast cancer bearing animal model utilizing the liposomes encapsulating taxol injected intravenously showed a better therapeutic effectiveness and a lower hemotoxicity than did free taxol [8]. Liposomes are, up till now, the most used nanocarriers for targeted drug delivery in the clinical setting.
Micelles: Micelles are colloidal dispersions constructed from amphiphilic molecules which tend to be ~20-80 nm in diameter. Their smaller size when compared to larger nanocarriers such as liposomes can limit their ability to carry a substantial dose of the chemotherapeutic agent to the tumor. The use of polymeric micelles for cancer treatment was first reported in the early 1980s by Ringsdorf and coworkers [9]. Micelles containing a folate moiety have been shown to be significantly more cytotoxic to ovarian carcinoma cells than non-targeted micelles [10]. In fact, folate has also been successfully used recently as a targeting ligand in micelles to deliver poorly water-soluble chemotherapeutics (either tamoxifen or paclitaxol) to colon carcinoma cells [11]. In addition, hyaluronic acid (HA)-paclitaxel conjugate micelles have recently been shown to be far more cytotoxic toward HA receptor over expressing cancer cells than for HA receptor deficient cells [12]. The clinical success based on passively delivering chemotherapeutics encapsulated within micelles in cancer treatment have made these nanocarriers particularly attractive candidates for future work involving a more active form of delivery.
Quantum dot: Quantum dots (QDs) are nanometer-size luminescent semiconductor nanocrystals. Their unique optical properties, such as high brightness, long-term stability, simultaneous detection of multiple signals and tunable emission spectra, make them appealing as potential diagnostic and therapeutic systems in the field of oncology. Yezhelyev et al. [13] reported the use of multicolor QDs for quantitative and simultaneous profiling of multiple biomarkers using intact breast cancer cells and clinical specimens and the comparison between the new QDs-based molecular profiling technology with standard western blotting and fluorescence in situ hybridization (FISH). In another study, Gao et al. [14] demonstrated the potential of QDs as a new diagnosis technology for metastasis prostate cancer.
Gold nanoparticles: Gold nanospheres (AuNPs) (also known as gold colloids) of 2 nm to over 100 nm in diameter can be synthesized by controlled reduction of an aqueous HAuCl4 solution using different reducing agents under varying conditions. The properties of AuNPs such as their size, charge and surface chemistry have been shown to affect the uptake of AuNPs into cells as well as their subsequent intracellular fate. In addition, effective drug delivery strategies must take into account the nature of drug-AuNP interaction (covalent/non-covalent binding) as well as the means of drug release following introduction of the drug-AuNP complexes to cells [15].
The utility of AuNPs as novel biosensors for the detection of tumor cells can be demonstrated through the use of a screen-printed carbon electrode (SPCE) coupled with a NP-based electrocatalytic method [16]. Huang et al. [17] have described two methods for tumor targeting: the first involved conjugation of AuNPs to polyethylene glycol (PEG), and the second involved conjugation of AuNPs with specific antibodies which bind unique biomarkers expressed on tumor cells. PEG prevented AuNPs aggregation and lengthened their retention time in blood. This facilitated the preferential accumulation of AuNPs in tumor cells over healthy cells because of the elevated permeability of poorly differentiated blood vessels around tumors following angiogenesis (Figure 3), as well as the decreased clearance rate caused by the deficit of functional lymphatic vessels in tumors [18].
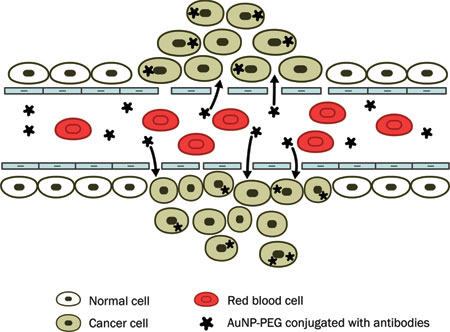
Figure 3: Schematic diagram showing accumulation of ligand-targeted gold nanoparticles conjugated with anticancer drugs in cancer cells mediated via extravasation of the gold nanocarriers through gaps in the endothelial cells (“leaky tumor vasculature”) [46].
Magnetic nanoparticles: Magnetic nanoparticles can be produced by a number of physical and chemical routes that differ in the final properties of the products. The common feature of all nanoparticle-based cancer therapies is the need of specific NPs for achieving the desired therapeutic effect. However, each diagnostic/therapeutic technique requires a different chemical or physical property of the particles involved, which depends on the specific function played by the NPs in that therapy (e.g., vector, porous receptacle, heating agent, magnetic moment carrier, etc.). Sometimes the particle function is activated using an external agent (magnetic fields, light, radiation, etc.) that interacts with the NPs. Therefore the requirements for NPs as biomedical agents span a broad range of novel materials, synthesis strategies, and research fields (Table 1). The appeal for using nanoparticles in selective tumor targeting is the potential to deliver a concentrate dose of drug in the vicinity of (or even inside) the target tissue, reducing drug exposure of healthy cells. This could be done by means of physical interactions, or passive/active targeting [19]. The other way to deliver drugs to any desired target involves the functionalization of the surface of nanoparticles with monoclonal antibodies or ligands to tumor-related receptors, taking advantage of the specific binding ability between an antibody and antigen, or between the ligand and its receptor [20, 21].
Carbon nanotubes: A Carbon nanotube (CNT) is a tube-shaped material, made of carbon, having a diameter measuring on the nanometer scale. As a group, Carbon nanotubes typically have diameters ranging from <1 nm up to 50 nm. Their lengths are typically several microns, but recent advancements have made the nanotubes much longer, and measured in centimeters. The shape of the CNT would allow these materials to enter the cell via different methods, such as passive diffusion across the lipid bilayer, or endocytosis, whereby the CNT attaches to the surface of the cell and is subsequently engulfed by the cell membrane. The hollow monolithic structure of CNTs and their ability to bind desired functional groups make CNTs promising drug carriers. They can be functionalized to be more water-soluble and serum-stable, with low toxicity at the cellular level [22, 23].
It has been suggested that CNTs could be used as nanocarriers for delivering drugs into the body via injectable routes [24]. It is beyond the scope of this article to describe all of them in detail, but they have been succinctly summarized in a series of recent reports (Table 2). Drugs can either attach to the outer surface of the CNT via functional groups or be loaded inside the CNT. Attachment of the anticancer drug to the outer surface of the CNT can be through either covalent or noncovalent bonding, including hydrophobic, π–π stacking, and electrostatic interactions [25-27].
Dendrimers: Dendrimers are nano-sized, radially symmetric molecules with well-defined, homogeneous and monodisperse structure consisting of tree-like arms or branches. The properties of dendrimers, in particular the synthetic ability to provide them with many different biological properties, along with their capacity to carry conjugated surface molecules or encapsulated guest molecules, make them immediately attractive as potential vehicles for drug delivery. Once a dendrimer carrying an encapsulated drug reaches the intended site of action, the guest molecule generally must be released to gain bioactivity. The observation that guest molecules could be liberated at different rates demonstrated that viable opportunities exist to tailor the release for either slow or rapid delivery (Figure 4).
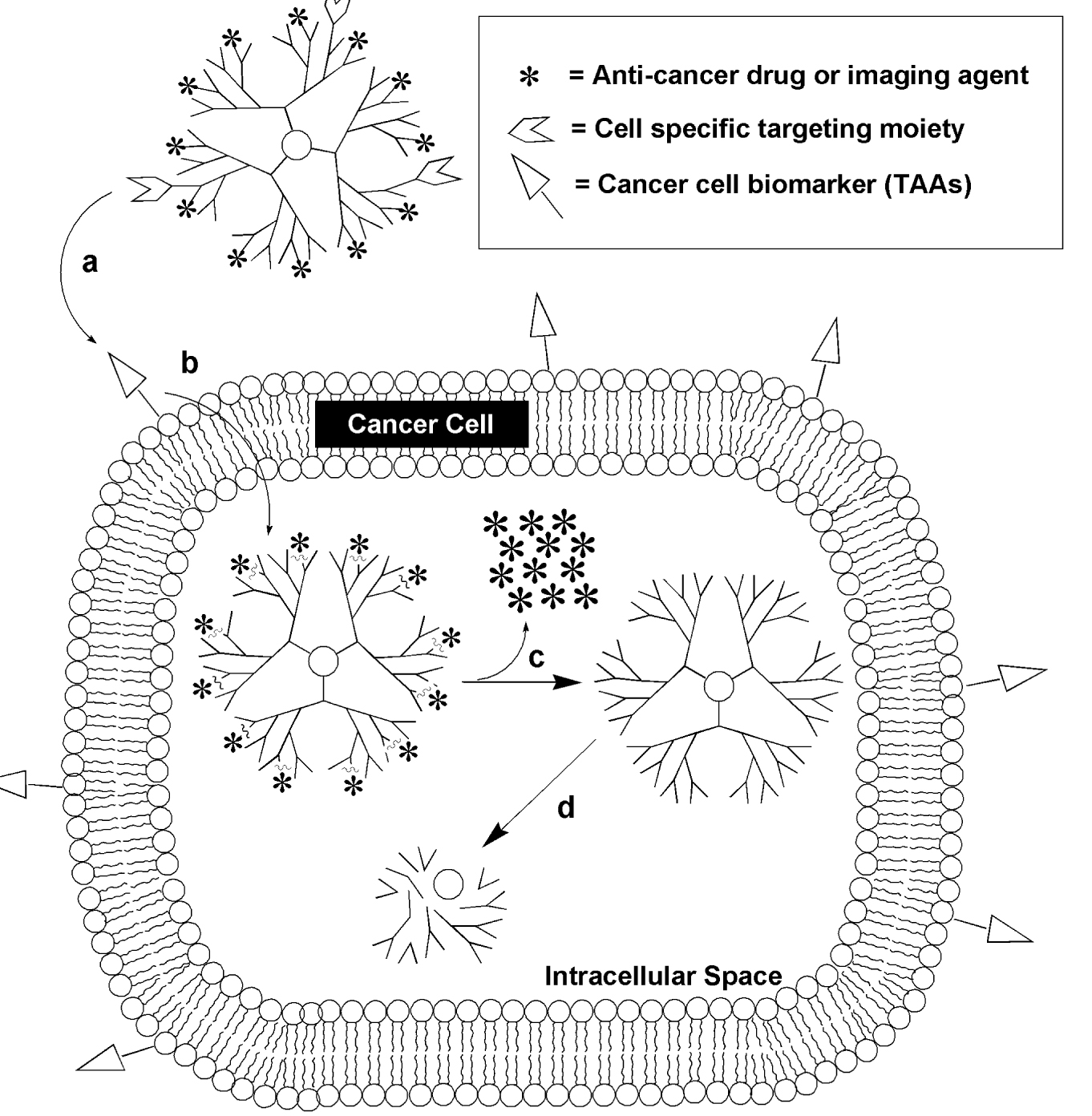
Figure 4: Requirements for dendrimer-based cancer-targeted drug delivery. (a) Dendrimers with multiple surface functional groups can be directed to cancer cells by tumor-targeting entities that include folate or antibodies specific for tumor-associated antigens. (b) The next step is intake into the cell, which in the case of folate targeting occurs by membrane receptor-mediated endocytosis. (c) Once inside the cell, the drug generally must be released from the dendrimer, which, for the self-immolative method, results in the simultaneous disintegration of the dendritic scaffold (d) (Srinivasa-Gopalan et al.[45]).
The Schluter group examined the impact of peripheral functionality on the cytotoxicity of MCF-7 breast cancer cells in vitro using low generation (G0, G1, and G2) polyamidoamine-like polymers [28]. Paclitaxel was conjugated to PEG or G4-PAMAM to compare the anti-cancer activity of the drug delivered by a linear or dendritic carrier [29]. Studies have shown that folic acid-conjugated dendrimers preferentially target tumor cells that overexpress folic acid receptors [30]. A recent study by Hong et al. explicitly quantified the binding avidity of multi-valent targeted G5- PAMAM containing different numbers of folic acid molecules [31]. The conjugates were prepared and evaluated against a cancer cell line (HeLa) and healthy cells (non-transformed mouse embryonic fibroblasts or MEFs) [32]. The importance of the high architectural control characteristic of dendrimers has been increasingly supported by positive outcomes from in vitro and in vivo studies. Studies have indeed demonstrated that a well-designed dendrimer structure can be potentially tuned simultaneously for desired biocompatibility, bioavailability, pharmacokinetics, and localized delivery of therapeutics to malignancies. Continued research in the area will bring compositions and architectures tailored for increasing specificity and efficacy towards the diagnoses and treatment of cancer in the clinic.
Nanoparticle platforms for Cancer Stem Cell targeted drug delivery
The intratumoral heterogeneity of cancer cells presents a major challenge to the development of effective cancer therapies. However, a growing body of evidence suggests that tumors may be driven by a small population of transformed stem-like cells with the ability to undergo both self-renewal and differentiation into the diverse cancer cell population that constitutes the bulk of the tumor [33, 34, 35]. Nanoscale drug delivery technologies offer fundamental advantages over contemporary small molecule pharmaceuticals used in clinical practice. These advantages include increased bioavailibity, extended drug half-life and reduced off-target toxicities [36].
Biomarkers of cancer
Identification of biological markers of cancer is a major area of research. Every cell type has a unique molecular signature, referred to as biomarkers, which are identifiable characteristics such as levels or activities (the abilities of genes or proteins to perform their functions) of a myriad of genes, proteins or other molecular features. Biomarkers have tremendous therapeutic impact in clinical oncology, especially if the biomarker is detected before clinical symptoms or enable real-time monitoring of drug response. Protein signatures in cancer provide valuable information that may be an aid to more effective diagnosis, prognosis, and response to therapy. More than 11 million people are diagnosed with cancer every year [37].
Alterations primarily in three main classes of genes viz., (proto) oncogenes, tumour suppressor genes and DNA repair genes collectively contribute to the development of cancer genotype and phenotype that resists the natural and inherent death mechanism(s) embedded in cells (apoptosis and like processes), coupled with dysregulation of cell proliferation events (Figure 5). There is a critical need for expedited development of biomarkers and their use to improve diagnosis and treatment for cancer [38]. Just recently, scientists have begun identifying microRNAs (miRNAs) as cancer biomarkers [39, 40]. In fact, miR-141 levels could identify prostate cancer patients with high sensitivity and perfect accuracy [40]. Peng et al. reported that a tailor-made array of cross-reactive sensors based on organically functionalised gold nanoparticles discriminates between breath Volatile Organic Compounds (VOCs) of healthy controls and of patients suffering from lung, breast, colorectal, and prostate cancers [41].
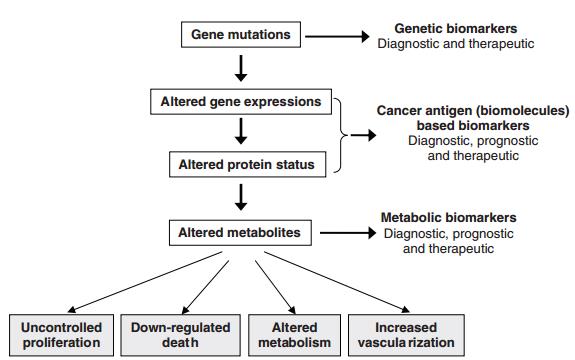
Figure 5: The process of carcinogenesis, showing opportunities of identifying biomarkers.
Nanomaterials: future drugs for cancer chemotherapy
During the past few decades, various chemotherapeutic agents, such as cyclophosphamide, fluorouracil, platinum-based compounds, anthracycline, hydroxycamptothecin and paclitaxel, have been designed and proved to be effective toward cancer cells. However, regrettably, these drugs are non-targeted to cancer, and thus serious side effects to normal cells or tissues are unavoidable [42]. Therefore, new drugs with selective cytotoxicity become an important research focus in cancer chemotherapy. Nanotechnology-based approaches are anticipated to provide a new breakthrough for targeting cancer cells and bypassing their multidrug resistance [43].
On the other hand, in the clinical cancer therapy process, anticancer drugs are simply employed to kill cancer cells. Unfortunately, nontargeted drugs may be rapidly and widely distributed in healthy organs and tissues. As a result, a high dose of anticancer drugs is normally needed to obtain favorable therapy efficacy. Moreover, the patients have to suffer from severe side effects or even from the drug toxicity far earlier than the tumor burden. Presently, these clinical difficulties have largely impeded successful cancer therapy.
Cancer detection
Conventional: Conventional detection of the cancer is done by observing the physical growth/changes in the organ by X-rays and/or CT Scans and is confirmed by biopsy through cell culture. However the limitation of this method is that it is not very sensitive and the detection is possible only after substantial growth of the cancerous cells. Often the treatment is also not possible once the cancer is in such an advanced stage.
Nanotechnology detection: As mentioned before, nanoparticles are of a few of nm and the cells are of the size of few microns. So NP can enter inside the cells and can access the DNA molecules/Genes and, there is a possibility that the defect in the genes can be detected. DNA molecules can be detected in their incipient stage. This could be possible in vivo or in vitro. It will be shown latter that NPs do show potential of cancer detection in its incipient stage.
Cancer treatment
Conventional: One of the treatment options is surgery. That is, remove the cancerous part. However, the limitation is that one loses the organ and the cancer may appear again. Further, the surgery is not possible for all types of cases of the cancer. Second option is radiation therapy. In this the cancerous cells are burnt by radiation of specific frequency band and the intensity. The limitation of this method is that even the healthy cells get burnt, cancerous cells burning is not uniform and the burnt part may become dead and non functional. The third option is chemotherapy. That is, cancerous cells are killed by drugs toxic to cells or by stopping cells from taking nutrients needed to divide the cells or stop the mechanism responsible for division of the cell. Normally a combination of drugs is given so that drugs affect all the three aspects of the cancer treatment. The limitation of this approach is that treatment is harmful to healthy cells, approach is gross and rarely successful if the cancer is in advanced stage.
Nanotechnology: Certain nanoparticles can be designed to absorb preferentially certain wave length of radiation and gets heated. Such a NPs if enters in the cancerous cell will burn it if irradiated by suitable wavelength radiation. This is kind of the analogue of radiation therapy. As mentioned before, nanotechnology can be used to create therapeutic agents that target specific cells and deliver toxin to kill them. The NP will circulate through the body, detect cancer associated molecular changes, assist with imaging release a therapeutic agent and then monitor the effectiveness of the intervention.
Conclusions
Nanotechnology is definitely a medical boon for diagnosis, treatment and prevention of cancer disease. A number of approaches for successful applications of nanomaterial-based cancer drugs will be possible because of their unusual characteristics. Areas of greatest clinical impact likely include novel, targeted drug-delivery vehicles, molecularly targeted contrast agents for cancer imaging, targeted thermal tumor ablation, and magnetic field targeting of tumors. However, there are still challenges to the development and application of nanotechnology platforms in cancer therapy, such as limited knowledge of the cancer cell physiology, small variety and poor functionalization of medical nanomaterials, and deficiency of clinical evaluation criteria. Nonetheless, with further advances in functionalization base on thorough understanding of the physiological features of cancer cells, nanotechnology platforms hold the promise of essentially changing the practice of oncology, allowing easy and effective targeted therapies. The clinical success of various nanocarrier constructs in cancer therapy have made these and similar systems promising drug delivery vehicles for future work aimed to further improve their overall drug delivery efficacy. Nanoparticles will likely serve as the norm rather than an exception in the majority of all areas of future conventional cancer treatments.
Conflicts of interest
Authors declare no conflicts of interest.
References
1. Feynman RP. There’s plenty of room at the bottom. Presented at the Annual Meeting of the American Physical Society, December 29, 1959, California Institute of Technology, Pasadena, CA. http://www.zyvex.com/nanotech/feynman.html.
2. Sanvicens N, Marco MP. Multifunctional nanoparticles—properties and prospects for their use in human medicine. Trends Biotechnol 2008, 26:425–433.
3. Schroeder A, Heller DA, Winslow MM, Dahlman JE, Pratt GW, et al. Treating metastatic cancer with nanotechnology. Nat Rev Cancer 2011, 12:39-50.
4. Cai W, Hsu AR, Z.-B. Li, Chen X. Are quantum dots ready for in vivo imaging in human subjects?. Nanoscale Res Lett 2007, 2:265–281.
5. Cai W, Chen X. Nanoplatforms for targeted molecular imaging in living subjects. Small 2007, 3:1840–1854.
6. Johannsen M, Gneveckow U, Thiesen B, et al. Thermotherapy of prostate cancer using magnetic nanoparticles: feasibility, imaging, and three-dimensional temperature distribution. Eur Urol 2007, 52:1653–1662.
7. Nombona N, Maduray K, Antunes E, Karsten A, Nyokong T. Synthesis of phthalocyanine conjugates with gold nanoparticles and liposomes for photodynamic therapy. J Photochem Photobiol B 2012, 107:35-44.
8. Reszka R, Brandl M, Fichtner I, Warnke G. Liposome-encapsulated Taxol, its preparation and its use. US patent 6090955, 2000.
9. Gros L, Ringsdorf H, Schupp H. Polymeric anti-tumor agents on a molecular and on a cellular-level. Angew Chem Int Ed Engl 1981, 20:305–325.
10. Kim D, Lee ES, Oh KT, Gao ZG, Bae YH. Doxorubicin-loaded polymeric micelle overcomes multidrug resistance of cancer by double-targeting folate receptor and early endosomal pH. Small 2008, 4:2043-2050.
11. Licciardi M, Craparo EF, Giammona G, Armes SP, Tang Y, et al. In vitro biological evaluation of folate-functionalized block copolymer micelles for selective anti-cancer drug delivery. Macromol Biosci 2008, 8:615-26.
12. Lee H, Lee K, Park TG. Hyaluronic acid-paclitaxel conjugate micelles: synthesis, characterization, and antitumor activity. Bioconjug Chem 2008, 19:1319-1325.
13. Yezhelyev MV, Al-Hajj A, Morris C, et al. In situ molecular profiling of breast cancer biomarkers with multicolor quantum dots. Advanced Materials, 2007, 19:3146–3151.
14. Gao X, Chung LWK, Nie S. Quantum dots for in vivo molecular and cellular imaging. Methods Mol Biol 2007, 374:135–145.
15. Duncan B, Kim C, Rotello VM. Gold nanoparticle platforms as drug and biomacromolecule delivery systems. J Control Release 2010, 148:122–127.
16. de la Escosura-Muñiz A, Sánchez-Espinel C, Díaz-Freitas B, González-Fernández A, Maltez-da Costa M, et al. Rapid identification and quantification of tumor cells using an electrocatalytic method based on gold nanoparticles. Anal Chem 2009, 81:10268–10274.
17. Huang X, Jain P, El-Sayed I, El-Sayed M. Plasmonic photothermal therapy (PPTT) using gold nanoparticles. Lasers Med Sci 2008, 23:217–228.
18. Liu Y, Miyoshi H, Nakamura M. Nanomedicine for drug delivery and imaging: a promising avenue for cancer therapy and diagnosis using targeted functional nanoparticles. Int J Cancer 2007, 120:2527–2537.
19. Neuberger T, Schöpf B, Hofmann H, Hofmann M, von Rechenberg, B. Superparamagnetic nanoparticles for biomedical applications: Possibilities and limitations of a new drug delivery system. J Magn Magn Mater 2005, 293:483-496.
20. Duguet E, Vasseur S, Mornet S, Devoisselle JM. Magnetic nanoparticles and their applications in medicine. Nanomedicine 2006, 1:157-168.
21. Sinha R, Kim GJ, Nie S, Shin DM. Nanotechnology in cancer therapeutics: bioconjugated nanoparticles for drug delivery. Mol. Cancer Ther 2006, 5:1909-1917.
22. Sahoo NG, Bao H, Pan Y, et al. Functionalized carbon nanomaterials as nanocarriers for loading and delivery of a poorly water-soluble anticancer drug: A comparative study. Chem Commun (Camb) 2011, 47:5235–5237.
23. Beg S, Rizwan M, Sheikh AM, Hasnain MS, Anwer K, et al. Advancement in carbon nanotubes: Basics, biomedical applications and toxicity. J Pharm Pharmacol 2011, 63:141–163.
24. Beg S, Rizwan M, Sheikh AM, Hasnain MS, Anwer K, et al. Advancement in carbon nanotubes: Basics, biomedical applications and toxicity. J Pharm Pharmacol 2011, 63:141–163.
25. Liu Z, Chen K, Davis C, et al. Drug delivery with carbon nanotubes for in vivo cancer treatment. Cancer Res 2008, 68:6652–6660.
26. Wu W, Li R, Bian X, et al. Covalently combining carbon nanotubes with anticancer agent: Preparation and antitumor activity. ACS Nano 2009, 3:2740–2750.
27. Li Y, Cousins BG, Ulijn RV, Kinloch IA. A study of the dynamic interaction of surfactants with graphite and carbon nanotubes using Fmoc-amino acids as a model system. Langmuir 2009, 25:11760–11767.
28. Fuchs S, Kapp T, Otto H, Schoneberg T, Franke P, et al. A surface-modified dendrimer set for potential application as drug delivery vehicles: synthesis, in vitro toxicity, and intracellular localization. Chemistry 2004, 10:1167–1192.
29. Khandare JJ, Jayant S, Singh A, Chandna P, Wang Y, et al. Dendrimer versus linear conjugate: influence of polymeric architecture on the delivery and anticancer effect of paclitaxel. Bioconjug Chem 2006, 17:1464–1472.
30. Quintana A, Raczka E, Piehler L, Lee I, Myc A, et al. Design and function of a dendrimerbased therapeutic nanodevice targeted to tumor cells through the folate receptor. Pharm Res 2002, 19:1310–1316.
31. Hong S, Leroueil PR, Majoros IJ, Orr BG, J.R. Baker JR, et al. The binding avidity of a nanoparticle-based multivalent targeted drug delivery platform. Chem Biol 2007, 14:107–115.
32. Lagnoux D, Darbre T, Schmitz ML, Reymond JL. Inhibition of mitosis by lycopeptide dendrimer conjugates of colchicines. Chemistry 2005, 11:3941–3950.
33. Charafe-Jauffret E, Monville F, Ginestier C, Dontu G, Birnbaum D, et al. Cancer Stem Cells in Breast: Current Opinion and Future Challenges. Pathobiology 2008, 75: 75-84.
34. Clarke MF, Dick JE, Dirks PB, Eaves CJ, Jamieson CH, et al. Cancer stem cells-perspectives on current status and future directions: AACR workshop on cancer stem cells. Cancer Res 2006, 66:9339-9344.
35. Prince ME, Sivanandan R, Kaczorowski A, Wolf GT, Kaplan MJ, et al. Identification of a subpopulation of cells with cancer stem cell properties in head and neck squamous cell carcinoma. Proc Natl Acad Sci USA 2007, 104:973-978.
36. Shapira A, Livney YD, Broxterman HJ, Assaraf YG. Nanomedicine for targeted cancer therapy: towards the overcoming of drug resistance. Drug Resist Update 2011, 14: 150-163.
37. Cho WSC. Contribution of oncoproteomics to cancer biomarker discovery. Mol Cancer 2007, 6:25. doi:10.1186/1476-4598-6-25.
38. Singha AK, Pandeyb A, Raib R, Tewarib M, Pandeya HP, et al. (2008) Nanomaterials emerging tool in cancer diagnosis and treatment: Digest Journal of Nanomaterials and Biostructures 2008, 3:135–140.
39. Chen X, Ba Y, Ma L, et al. Characterization of miRNAs in serum: a novel class of biomarkers for diagnosis of cancer and other diseases. Cell Res 2008, 18:997–1006.
40. Mitchell PS, Parkin RK, Kroh EM, et al. Circulating microRNAs as stable blood-based markers for cancer detection. Proc Natl Acad Sci USA 2008, 105:10513–10518.
41. Peng G, Hakim M, Broza YY, Billan S, Abdah-Bortnyak R, et al. Detection of lung, breast, colorectal, and prostate cancers from exhaled breath using a single array of nanosensors. Br J Cancer 2010, 103:542-551.
42. Schroeder A, Heller DA, et al. Treating metastatic cancer with nanotechnology. Nat Rev Cancer 2012, 12:39–50.
43. Wang L, Liu Z, et al. Docetaxel-loaded-lipid-based-nanosuspensions (DTX-LNS): preparation, pharmacokinetics, tissue distribution and antitumor activity. Int J Pharm. 2011, 413:194–201.
44. Tanaka T, Shiramoto S, Miyashita M, Fujishima Y, Kaneo Y. Tumor targeting based on the effect of enhanced permeability and retention (EPR) and the mechanism of receptor-mediated endocytosis (RME). Int J Pharm 2004, 277:39–61.
45. Srinivasa-Gopalan Sampathkumar, Kevin J. Yarema. Dendrimers in Cancer Treatment and Diagnosis. Wiley-VCH Verlag GmbH & Co 2007. 46. Lim ZZ, Li JE, Ng CT, Yung LY, Bay BH. Gold nanoparticles in cancer therapy. Acta Pharmacol Sin 2011, 32:983-990.